Leicester helicopter crash pictures: Latest horrific images outside King Power Stadium LEICESTER CITY owner Vichai Srivaddhanaprabha's helicopter crashed outside the King Power Stadium last night. And images of the crash site has emerged this morning. Mr Srivaddhanaprabha is understood to have been in the helicopter that left the King Power Stadium pitch at around 8.30pm on Saturday. Leicester had played out a 1-1 draw with West Ham in the Premier League's late kickoff.
Leicester are yet to release a statement on the incident this morning but club officials have arrived for a meeting. And images of the crash site have started to emerge as the day breaks over the city. A tarpaulin sheet appears to be covering part of the helicopter. The area of the car park where the chopper landed has been cordoned off. More to follow… A helicopter belonging to Leicester City's chairman has crashed outside the King Power Stadium.
The aircraft had just taken off from the pitch at the east Midlands' club and made it over the stand en route to London before it crashed into a car park outside the stadium. LATEST - HORRIFIC DETAILS EMERGE ABOUT TRAGIC CRASH Eyewitness accounts said the helicopter went into a tailspin before slamming into the concrete. It did not burst into flames immediately but was soon engulfed in a fireball.
It is unknown if Leicester City's chairman Vichai Srivaddhanaprabha was on board. The Thai businessman attends all of Leicester's home games and regularly leaves the ground in his helicopter. Speaking from outside the ground tonight, Sky Sports man Rob Dorsett said: "It crashed into the car park just a couple of hundred yards outside the stadium before bursting into flames. "The emergency service reacted very, very quickly.
There were police, fire brigade and ambulances here because of the game anyway. "They got to the scene very, very quickly and put the fire out after around 25 minutes. "By that time I'd managed to walk around the stadium and had seen the flames and smoke myself. "There were plumes of huge grey smoke pouring out of the wreckage outside the car park, outside the stadium.
"Now we need to stress we don't know who was on board that helicopter. "It holds, I know for a fact, 20 people. I have seen it take off many times from King Power Stadium with various dignitaries and members of the board after a game to take them home. "We don't know how many was on board this evening.
"The latest we have been told - and these are unconfirmed reports I must stress - is that Aiyawatt Srivaddhanaprabha, the vice-chairman of Leicester City, was not on board. He was not at the game, I'm told. "It's clearly a tragic situation, a very tragic accident, and one that's unfolding as we speak right now." While on Match of the Day commentator Alistair Mann told host Gary Lineker: "Those of you who have been to Leicester over the last few years know it's fairly common place for half an hour after the game, it goes up and takes him to wherever he wants to go.
"This was the situation again this evening. It's a common situation but within barely seconds of it taking off and going above the stand over the top of the ground, it appears to have reached some difficulties and has come down. "The impression I get is beyond the car parks that are immediately around the vicinity of the stadium but fairly nearby nonetheless. "It's come down, apparently there was smoke initially, then a pop and it seems to have become engulfed in flames.
"Look, we don't know who is on board at this stage and we don't know for sure those who got on to the helicopter, but ordinarily it takes the family, we can't confirm if the chairman was on, we can't confirm if any of his family members were on, I'm led to believe it's normally a two-person crew. "I believe that was the case this evening but we really don't know for sure who was on board. That appears to be what happened.
"Because there was a game on tonight, the emergency services were in the vicinity so there was a very quick reaction and lots of police and ambulances and so on very quickly on to the scene to control the situation. "It is a very serious situation but we really don't know the extent of it just at the moment." Leicestershire Fire and Rescue Service's Assistant Chief Fire Officer Andrew Brodie tweeted regular updates on the situation overnight.
He wrote at 9.48pm: "IMPORTANT: Energency Services are dealing with a significant incident @lcfc King Power stadium. It's essential you stay away and allow 999s free movement.
More info will follow." At 1.13am he delivered a second update which read: "Leaving @leicspolice HQ after multiagency strategic meetings following helicopter incident. Clearly serious and tragic, please don't speculate on cause or who may be involved.
Think of families, friends, responders, and @LCFC and their fans." "Just visited the @LeicsFireRescue @leicspolice @EMASNHSTrust crews still on scene of the helicopter crash @LCFC. Honestly, your police, ambulance and fire responders are magnificent, caring and dedicated. Don't ever forget it," he added just before 2am.
For more infomation >> Leicester helicopter crash pictures: Latest horrific images outside King Power Stadium - Duration: 6:37.-------------------------------------------
Lovely House Warm Atmosphere Surrounded by Lawns and Flowers - Duration: 3:45.
Lovely House Warm Atmosphere Surrounded by Lawns and Flowers
-------------------------------------------
Ask Fausto #2 - How to visualize magnetic fields and fieldlines - supermagnete - Duration: 5:20.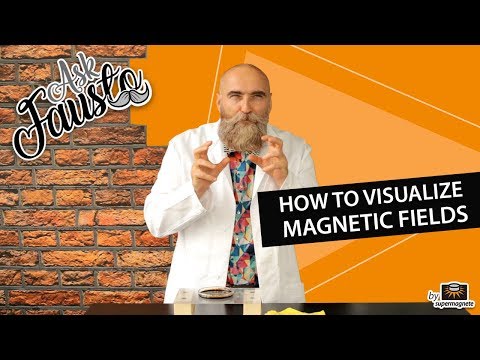
-------------------------------------------
Genes, Cognition, and Human Brain Evolution - Duration: 51:03.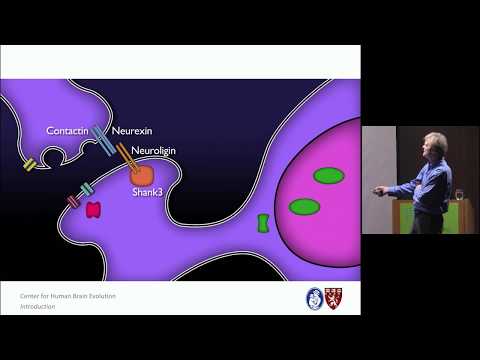
Dr. Christopher Walsh, like many Harvard professors,
has many, many titles and honors and everything else.
So he is the Bullet Professor of Pediatrics and Neurology
at Harvard Medical School, the chief
of the Division of Genetics and Genomics
at Boston Children's Hospital, an investigator of the Howard
Hughes Medical Institute, and an associate member
of the Broad Institute as well.
He completed his MD and PhD degrees
at the University of Chicago, neurology
residency and chief residency at Massachusetts General,
and post-doctoral training in genetics
at the Harvard Medical School.
In 1993, he became assistant professor of neurology
at Harvard and Beth Israel Deaconess Medical Center,
and he has been the Bullet Professor since 1999.
He moved to Boston Children's Hospital
in 2006, where the Division of Genetics and Genomics
diagnoses and treats children with diverse, rare,
and orphan diseases, is undertaking
more than a dozen clinical trials
of innovative medical and gene therapies
for genetic disorders.
His research has focused on the development, function,
and evolution of the human cerebral cortex,
pioneering the analysis of genetic diseases
that affect the developing brain by fostering
worldwide collaborations with physicians and families.
His lab has identified genetic causes
for dozens of brain diseases of children associated
with autism, intellectual disability, seizures,
and cerebral palsy.
He has discovered that some of these disease genes
were important targets of the evolutionary processes that
shaped the human brain.
2017, he inaugurated the Allen Discovery Center
for Human Brain Evolution at Boston Children's Hospital
and Harvard Medical School, collaboratively
with Doctors Michael Greenberg and David Reich.
And of course, we are going to have
David Reich speak in a few weeks as part of this series as well.
And this Center brings together brain science
with evolutionary genetics to search
for the key changes in the genome that endow humans
with our unique abilities for language, art, culture,
and science.
He has, of course, been recognized by research awards
from many different places that I'm not
going to list because really, you
want me to get off the stage and for him to get on the stage.
But I would like to mention that he
is an elected member of the American Association
of Physicians, the National Academy of Medicine,
the American Association for the Advancement of Sciences,
the American Academy of Arts and Sciences,
and the National Academy of Sciences.
So I feel like phew, and we're really looking forward
to hearing you speak.
Thank you.
[APPLAUSE]
Well, thank you so much, Jane, for inviting me to come
speak here.
I'm really thrilled to come over and talk
to a general audience about a lot of the enthusiasm
that we have about trying to understand
those genetic changes that make us human,
and I really appreciate your generous introduction
and the fact that you did it in front
of one of two members of my family, so--
[CHUCKLING]
But anyway, so I'm delighted to have a chance
to chat with you all for the next 45 minutes
or so about at least how we think about the evolution
of the human brain.
And I'd like to introduce this Allen Discovery Center
for Human Brain Evolution.
As Jane mentioned, it's a collaboration
between our lab and David Reich and Michael Greenberg's lab
at the Department of Neurobiology,
and also, Alice Lee and Ryan [? Dome. ?]
And we'd be nowhere without Soha Ashrofi, who's
our program manager.
You can visit our website to learn more about the center,
and I'll be telling you in the second half
of the talk a little bit about what we're
trying to do with the Center in terms of some of the science
programs.
So I'm trained, as Jane said, as a developmental neuroscientist
in the lab.
And then, as a neurologist, I never really
thought I would be an evolutionary biologist,
but you'll get a sense of how I stumbled into that field
over the course of the talk, I hope.
So I'll just start out by saying none of us
see the world the way it really is.
Our view of the world is a creation of our sense organs,
and every species sees a completely different world.
They perceive a completely different world,
and you can illustrate that fact by thinking about how
a shark sees the world.
So a shark hears its prey almost a mile away,
and it smells its prey more than 100 meters away,
and it can actually feel its prey up to 100 meters away.
I have no idea what that would feel like, but for the shark,
they have sensory organs in the outside of their skin.
And so the sense of touch for a shark
is a sense that acts at a distance.
It can actually perceive things that are, again,
far enough away that we can't even see them in some cases.
And then they can perceive, actually,
the electrical activity of its prey up to 50 centimeters away
through the water, and I have no idea what that would even
feel like, to be able to perceive
the electrical activity of someone else in this room.
And then, actually, a shark can taste its prey
before it even gets it into its mouth,
because it has taste buds all over the outside of its face.
And then, the shark has such terrible vision
that it only finally sees its prey
as it's going into its mouth.
And so this is a completely different world
in terms of-- try to picture yourself as a shark,
thinking about this blind thing where you're just
responding to senses or odors or sensation at a huge distance.
So every species is like this.
Every species has its own set of sensors, its own brain,
and perceives a completely different world.
And in fact, of course, even different humans
perceive a different world.
We know that some people are colorblind and don't perceive
the same colors that we do.
We know that many animals don't see color
at all, similar to some humans.
We know that people who are congenitally blind--
their brain builds a picture of the world based
on completely different information than most of us
take for granted because we're such highly visual species.
And so every animal has its own sort of brain,
and all of these different brains
are the result of ruthless rules of evolutionary selection
that have acted over millennia and that
have formed and shaped that nervous system so that it
processes just the kind of information
that that species has to, and basically then ignores
things which are not important.
So how do we understand our particular form
of the human brain?
Unlike all of these other animals,
we started by our ancestors leaving footprints
in the Earth, and now we are leaving footprints on the moon.
And so how is it that we got to be
so different than these other species in terms
of these capabilities?
And so that's really what we're trying
to get at when we study this problem of human brain
evolution.
So just to outline what I'll be talking about,
this is a problem that there's no threat
that we'll have it worked out any time soon.
But I'll try to give you a sense of how
we think about the problem scientifically,
and then a few examples of how we've
studied the problem, a couple of examples of stories
where the evidence seems to be fairly strong of having found
particular genes or genetic changes
that seem to have had roles in evolution.
And then, as I said, I'll close by talking a little bit
about the goals of the Allen Discovery Center
and how we hope to really catalyze
much more rapid discovery in this area in the years ahead.
So again, I'm not an anthropologist, but I do
want to just orient everyone relatively briefly
by reminding people of the sort relatively recent evolution
of human ancestors over the last couple of million years,
and remind you that we separated from chimps, our closest living
relatives, something like 6 million years ago.
And then, early hominids went through a series
of various shapes and sizes.
But like I say, I'm not capable of discussing
in any great detail.
I'll mention three-- homo sapiens, of course-- us--
as well as Neanderthals, and then
a third, relatively recent but extinct human forebear,
the Denisovans, which does not have a skull showing here
because, in fact, Denisovans is an extinct human forebear,
but we actually only know about their existence from their DNA.
We have a complete DNA sequence of their genome,
but no one has yet identified a bone or a skull which
is unambiguously Denisovan.
So we know they were there, but we just
don't yet know exactly what their form was.
So again, just to give a brief, cartoonish view of relatively
recent evolution to orient people,
Homo erectus lived about a million
to 2 million years ago in Africa.
And then, Homo erectus spread out of Africa into Europe.
Homo erectus gave rise to Neanderthals, Denisovans,
and modern humans.
So all of this happening in Africa--
and then, Neanderthals and Denisovans actually
ruled the entire Eurasian continent
for about 150,000 years.
That's three times as long as modern humans have
ruled the Eurasian continent.
So in fact, they have been around much longer
than we have--
for all we know, much longer than we will be.
And then modern humans, as a species--
Homo sapiens, as we recognize them--
again, first came into being in Africa at least
200,000 years ago.
Best estimates are about 300,000 years ago.
And then, from Africa, spread into Eurasia, where they met.
And actually, there's strong evidence
that they interbred with Neanderthals and Denisovans.
You may have heard that Europeans all
carry-- about 2% or 3% of our DNA
is derived from Neanderthals.
Asians carry a certain amount of Denisovan DNA,
based on this ancient interbreeding
between these ancestors.
But eventually, modern humans displaced
Neanderthals and Denisovans.
We don't exactly know how.
These numbers refer to the numbers of thousands of years
ago that humans hit the Arabian Peninsula or hit Asia
or crossed the Bering Strait into North America
and eventually colonized the entire world.
So the general question is, then,
how does evolution work on the brain?
And this turns out to be--
just the question itself turns out
to be kind of a complicated one.
Of course, it goes without saying that DNA
is the canvas of evolution.
It is the canvas on which evolution is recorded and then
is packaged and transmitted to the next generation.
And so that part goes without saying.
Comparing DNA sequences between different species,
then, you can identify parts of the genome
or particular sequences that might
be specific to each species.
And so we can then identify parts
of the genome that are specific to humans,
and I'll tell you about some of them.
An evolution occurs by natural selection, of course,
and natural selection then chooses
the genomes that are more fit.
So basically, ultimately, evolution of the brain
must ultimately reflect changes in DNA sequences
that regulate the way the brain develops
or the way the brain is formed or the way it functions.
But the difficulty comes in trying
to understand exactly how DNA defines our behavior when
it comes to the brain, because the purpose of the brain
is, in fact, to dynamically change based on events.
Hopefully, when you leave this room,
your brain will have been permanently
changed by some little thing that I said or somebody
else said.
And so, in fact, the human brain--
what I just told you is that once
humans emerged from Africa, they basically
filled every single environment in the entire planet,
and so they were endowed with a brain that
makes them immensely plastic.
And as far as we know, the genome
didn't necessarily change a great deal.
And so these changes, this plasticity, this learning
is inscribed not in our DNA, but in fact,
in changes in the neuronal connections in our brains,
between brain cells.
These connections are called synapses,
and the neurons between them then changed ever so slightly.
And so our genome in some way defines us,
but in fact, it doesn't make us.
There's this dynamic paradox between
this genetic determinism and this tremendous neuronal
flexibility that, in fact, is part and parcel of being human.
So just to kind of review, this is a picture
of a couple of brain cells.
And basically, what they do is they communicate electrically.
They fire these things called action potentials that
go down one neuron.
And then, in fact, signals are transmitted from one neuron
to the next by chemical neurotransmitters,
and then the brain learns by virtue of the fact
that when the activity in one neuron
is correlated with the activity of another neuron,
then the connections between them become stronger.
And so these synapses then have the ability
to change or be modified based on patterns of activity,
because those patterns of activity
reflect what we're exposed to and what we consider important.
And so you would think that a lot of the genes that
might have changed to make humans so special
might have to do with some of these genes
that encode proteins that make up parts of the synapses,
like these things, these receptors that here are
connecting the one neuron shown here, the presynaptic neuron,
to the postsynaptic neuron, or perhaps some
of these signaling molecules.
And here's the names of some of these molecules
that are in the neurons themselves.
So the other thing is that then, the origin story
of how evolution worked on our genome to make us human also
has to interact with another origin story, which
is the story of how an embryonic epithelium prenatally becomes
transformed into this neural network that produces
intelligence, creativity, social behavior,
and the scientific method.
And really, this is where I got my start
as a developmental neuroscientist and neurologist,
is studying abnormalities of this development.
So this shows a cross-section of a developing
brain of a laboratory animal.
This happens to be a ferret, which
I'll tell you a little bit more about, a little carnivorous
animal that likes to chase mice and chase them into holes
and eat them.
And so this is just an example of what
a mammal looks like during the time when the brain is
being put together.
And you see these very long, little fibers.
These are called radial glial fibers.
These are the stem cells that put the brain together.
This is a cartoon of what it looks like.
There are these very primitive stem cells shown in red,
and then, these are slightly more
mature dividing cells, and then the neurons
after they're formed.
They migrate away from where the cell bodies of the progenitor
cells are, and the neurons collect here
on the outer surface of the brain.
And so anyway, we have to then understand,
how do the genes fit into this pattern of brain development?
So to get back, then, to the time course of evolution,
there are three key phases of human brain evolution.
Somewhat more than 200,000 years ago, we
see that the size of our human forebears
tripled compared to that of the chimpanzee,
and this was fairly rapid.
The x-axis here is millions of years ago.
So this is minus 1 million, 2 million, 3 million, 4 million,
5 million, 6 million, and back to 10 million.
And you can see the expansion of the volume of the brain
from about 1,500 centimeters, which is where we are today,
to about 500 centimeters cubed, which was roughly
where chimpanzees would be.
So this was a very rapid change in evolutionary terms.
And then, between 200,000 to 50,000 years ago,
we see the development of modern human behavior.
50,000 years to the present, we see,
then, changes in different groups since their separation
to different parts of the world.
But I think, again, it's a huge question.
How exactly do we define when humans became modern?
How do we define what aspects, based on the fossil record,
the anthropological record--
how do we know when a pre-human existed
with the sort of capabilities that we
think of as characteristic of ours today?
And here's an example that illustrates that paradox.
This is a cave painting from about 5,000 to 10,000 years
ago.
This happens to be-- actually, this
is a watercolor copy of a cave painting from Zimbabwe,
and you see this highly abstract and apparently simplified
pattern of drawing.
And so we tend to look at that and we think,
well, they weren't very good artists back then, were they?
But then, of course, if you see these by Giacometti,
you say, well, this is obviously a deliberate abstraction
created for artistic effect.
And so the question is, what do we really
make of these paintings, and to what extent
are they as primitive as we might be easily to interpret?
And the reality actually is that Giacometti was, in fact,
influenced by those cave paintings
to develop his unique style because in fact,
those cave paintings were described just when Giacometti
was in his formative years.
OK, so next, I'll tell you about some
of the sorts of things that can happen to the genome that
can underlie evolution.
Basically, we think of evolution as occurring
by three general mechanisms in the genome.
There's only so many things that can happen to a genome.
And so the simplest thing to conceptualize
is that you can add a new gene to the genome.
So this is a picture of a genome, of a single cell.
These are the 46 chromosomes that
make up our genetic material.
Each of them, when you stain them like this,
they have little stripes.
These are the two chromosome ones,
which are named chromosome 1 because they're the largest.
And then you see that the chromosomes are numbered
in descending order of size.
So you can then take a chromosome here and take
a closer look at it, and you can go
on a website to see what your genome actually looks like.
So this is a schematic of a chromosome here.
You can see those same black and white stripes
that highlight the chromosome.
The red box is highlighting this particular segment
of the genome that then is shown at higher magnification.
And then, these blue things are actually genes.
They each have little names, typically three or four letters
or something like that.
Here's one gene.
Here's another gene.
Here's another gene.
There's spaces where there's no gene in there,
and so the simplest thing you can imagine
is that you just add a new gene to the genome.
Now, this turns out to be a relatively rare event
because there aren't just genes sitting around on the table
that nature can plug in.
Genes generally have to come from somewhere,
and they're complicated things that have to--
they have to be so precisely organized
so that they encode a protein.
But so the more common way in which gene addition will happen
is actually by the duplication of an existing gene,
and then tweaking it a little bit
so that it does something slightly different,
and I'll show you an example of that.
And so when you see duplications of a part of the genome
or a loss of the part of the genome,
we call them copy number variants
because it's a change in the copy number of the genome.
So this is what a normal chromosome would look like,
and I've just highlighted four segments of the genome labeled
A, B, C, and D. You can have a deletion that removes.
So these are the two homologous pairs of the same chromosome,
right?
We have two copies of each chromosome.
We have two copies of chromosome two,
two copies of chromosome one.
In this case, one of those two copies has lost the segment C,
or you can have an insertion where segment C is now
duplicated.
And so what this does is it duplicates
all of the genes in that segment,
and that segment might have 10 genes in it.
It might have 30 genes in it.
Might be a segment similar to the one that I just showed you.
And so here's a segment of the genome in the human genome that
happens to be a particularly fragile place,
and it's subject to frequent duplication,
actually, as a cause of medical disease.
And so this is chromosome one laid on its side,
and there is a segment on it here highlighted,
chromosome 1Q21.1.
And this actually is blown up, then,
and you can see there are several copies
of this gene called NOTCH2N.
There's an A and a B and a C copy.
All of these genes were originally
derived from an initial forebear gene called NOTCH2.
We have to give genes fancy names.
NOTCH happens to be named after a fly
because the gene was first found in a fly,
and when it's mutated, it causes a notch
in the fly's wing, which is probably what was first
recognized a century ago.
And then, of course, we found out
that all species have a NOTCH gene,
or virtually all eukaryotic species
have a-- or not eukaryote, but vertebrates and invertebrates.
Most animals and flies have a NOTCH gene.
So humans actually have several.
There is NOTCH2, and then that got duplicated several times
over.
This particular part of the genome actually,
though, in humans tends to vary a lot.
And in fact, you can have a deletion of this large segment
here, and that causes a disease.
That is associated with a higher risk of schizophrenia
and also makes the brain small.
And then, other patients can actually
have the corresponding duplication
of that same region, and the duplication
is associated with a big brain but also
predisposes to autism spectrum disorders.
And it's a whole other talk to tell you how that might happen,
but there are these reciprocal deletions and duplications,
suggesting that this part of the genome
actually might have something in it that's regulating
the size of the brain.
And as I just told you, the size of the brain
is very important in recent human evolution.
And this just shows what microcephaly looks like.
Micro means small.
Cephaly just means brain.
So this is a small brain.
This is a big brain.
And you can see that in this case,
the brain is smaller than is normal here,
but otherwise, looks fairly normally patterned.
It has all the usual folds that we call gyri and sulci, which
are just fancy Greek words for hills and valleys, that
characterize the outer surface of the brain.
And you can see over here, this is the circumference measured
around the head of kids.
And kids that have deletions-- their head circumference
is small.
Kids that have the duplication-- their head size is enlarged.
And so this is a part of the genome
that was recognized medically as regulating
the size of the brain.
It also contained this brain NOTCH,
which had been known to regulate stem
cells in many different parts of the body--
the wing as well as the brain, because it turns out NOTCH
is expressed in many different tissues.
And so recently, two groups, one at Seattle,
led by Evan Eichler, and another group in Europe, actually
started looking more carefully at NOTCH and saying,
well, hey, the size of the brain is important in evolution.
Might this NOTCH gene be part of that?
And so they studied the evolutionary structure
of this NOTCH2 area, and it turns out
that originally, orangutans and other great apes
only had a single copy of NOTCH2.
And then, in the lineage leading to humans,
it duplicated to create these NOTCH2NL things.
And then, in fact, on the human lineage,
there were further duplications that created
NOTCH2NL versions A, B, and C.
So in fact, humans have multiple different copies of NOTCH
as their brain got bigger and bigger, whereas again,
the great apes with smaller brains
have fewer copies of this.
And in fact, the same group did a series of studies
to show that this NOTCH2NL regulates the stem
cells in the brain and actually then helps
tell them to keep proliferating to make a bigger brain instead
of to stop proliferating, which is what
you have to do to make neurons.
So now that I've given you a course on genetics
and evolution, I'll give you a short course on neuroscience,
tell you a little bit about how stem cells in the brain
make neurons.
And so this is then a schematic of a fetus to--
again, similar to that picture that I showed you
that shows these stem cells, which
are along the ventricle, which is
a hollow area deep in the brain.
And then the cerebral cortex develops from the lining
of that hollow tube.
And again, the stem cells are those elongated things
on the inner surface, and they give rise, then--
they have these elongated processes.
This movie runs a little slow.
But the NOTCH2 gene is expressed in these progenitor cells.
Progenitor cells are giving rise to these bubbles,
which are representative of postmitotic neurons.
Neurons in your brain don't divide,
and they're not like your skin, where they'd
regenerate if you get a cut.
And so the number of neurons that you
have when you're born, with a few exceptions in a couple
of parts of the brain, are basically
the same number of neurons that you're always going to have.
And so these neurons are born prenatally
during the first couple of trimesters
of fetal development, and they become post-mitotic.
They migrate to the outer surface of the brain.
And as you collect more and more of them,
the cortex gets understandably larger.
So the NOTCH gene, then, is expressed in these cells--
not in the neurons, but in the cells that give
rise to the neurons, and it's basically a switch
that tells those stem cells.
Stem cells are great for making the brain,
but you can't think with your stem cells.
You only think with your neurons.
The neurons are the things that have synapses.
So at some point, if you're going to be smart,
the stem cells have to stop dividing
and give you these neurons that can then
develop these sophisticated connections and synapses.
So NOTCH, then, seems to work in these stem cells.
And this is another realistic picture, again from ferrets,
using a bunch of different colors.
The stem cells are down here, shown schematically
in this cartoon, and they give rise
to the neurons that migrate up here.
They stop migrating and they make their connections.
And so it seems then that this NOTCH, this increase
in the number of copies of NOTCH,
seems to be able to then regulate
the size of the brain evolutionarily
as well as medically.
And so then, it seems like humans
have just the right number of copies
of this NOTCH2 because if we have too many,
we have this problem with the brain being too big,
and that causes cognitive problems.
And if we have not enough, that causes problems as well.
So a somewhat more common mechanism of evolution
is to take a gene which is already in there
and tweak it a little bit by changing
the sequence of the gene.
Now, as you know, DNA encodes RNA, and then the RNA codes
for a particular sequence of amino acids
that generates a protein.
And so the biochemical patterns of a protein
are defined by its amino acid sequence.
If you swap one amino acid for another,
it can change the shape of the protein,
and hence, its biochemistry.
So this is, again, an important mechanism of evolution.
And again, one of the best characterized examples of this
is a gene that regulates the size of the brain.
So in this case, again, we have a gene here.
You blow it up, and we can look more carefully
in detail at the sequence.
Here, the amino acids are each abbreviated by a single letter.
And then, if you introduce a few changes in that amino acid
sequence, that can then change, as I say,
the biochemistry of the protein in a way.
And so then, in this case for evolution,
nature doesn't have to invent a completely new gene,
but it can endow an existing gene with new properties.
And so again, we get back to this rapid change in brain size
in relatively recent evolution.
And this is actually where I stumbled
into studying evolution now more than 10 years ago, when
we worked with Jeff Woods to identify
one of the first genes which, when
completely disabled in humans, causes microcephaly.
So this is just a single gene that regulates
the size of the brain.
This gene is called ASPM--
again, named after a fly gene.
Abnormal Spindle-- A-S-P--
is the fly gene, and M--
we renamed it ASPM for Abnormal Spindle Microcephaly.
And so when we first cloned this gene,
we noticed a bizarre thing about it--
that the actual size of the encoded protein
was way larger in humans than it was in fruit flies, where
ASP had first been identified.
And then, the size in the mouse was sort of in-between.
And in fact, the size in the worm
was even tinier than in a fruit fly.
So it looked like the actual encoded protein
got bigger and bigger over the course of evolution.
And so we wanted to then test the idea that it might actually
be a targeted evolutionary selection,
and you can't really do that between widely divergent
species, and so we did it between more closely
related species-- just among the great apes.
And there's a statistical test you
can apply to this that looks at how common it
is that an amino acid gets substituted, because most
of the time, evolution has created genes exactly the way
evolution wants those genes to be,
and any change in the sequence of the gene is usually trouble.
It's usually a mutation and typically causes disease,
and that's, in fact, the sorts of mutations
that were causing this.
But every once in a blue moon, a little tweak
in the sequence of a protein might not be damaging
and might improve it in some way but this
is much less common than for a change to be damaging.
So for a normal protein, the sequence of that protein
is carefully guarded, and so we can
look at changes in the DNA sequence
either that change the sequence of the protein
or that don't change the sequence of the protein.
For a normal gene, the vast majority
of changes in the DNA conserve the protein
sequence, but for this part--
and so we see a ratio of those two that's less than one.
For this particular gene, there's
an excess, a ratio greater than one
of changes that actually alter the sequence of the protein.
And this is quite a rare event, providing strong evidence
that, in fact, this gene was changed in some way, very
possibly with the increase of the size of the brain
in recent evolutionary time.
Now, the first thing you want to do as a biologist--
when you identify a gene like this, you say,
wow, this is the mechanism of evolution.
Let's try to get at the cellular biological mechanism of how
this ASP thing works.
And so what we did was we created a gene mutation in mice
to try to model what the gene might be doing in humans.
And here you see the comparison between a normal human
and a human that has an ASPM mutation.
Again, the brain is reduced up to 2/3 of its size--
a very small brain, but normally patterned.
Well, you knock it out in mice, and almost nothing happens.
You can't really tell which are the mutants
and which are the wild types, so there's about a 10% reduction
in the size of the brain.
And so this is kind of frustrating,
and the point seems to be that, in fact, the gene is
doing different things in these different species.
Now, when you actually look at the stem
cells in the human brain and you compare them to the mice,
in fact, even the progenitor cells are very different.
It just looks like the mouse brain is being put together
in a very different way.
The mouse has two types of stem cells-- the red
and the yellow ones.
And then, ferret is an example of a more common sort
of mammal pattern.
They add these green progenitor cells
that are long like the red ones, but not
quite all the way up from the very top to the very bottom.
In humans, these green cells, called Outer Radial Glial
cells, or ORGs--
they are the most abundant progenitor of all in the brain.
Many of my colleagues and maybe [INAUDIBLE] a little bit
think that these brains might, to a certain extent,
be the seed of the soul because they
become so abundant in humans.
They seem to be doing something extremely important,
and yet they are absent in mice.
And here, you see, actually, these--
the stem cell zone down here has these red type cells,
and that's about the same in mice and in ferrets
and in humans.
In fact, the size of that is maintained about the same,
but this outer zone that contains
these green cells, in fact, becomes a little expanded
in ferrets compared to mice, and then
usually expanded in humans compared
to actually any other species.
Virtually all mammals are very different than rodents
in this way.
Now, I told you that, in fact, the mouse and the human
have fairly different sequences of this ASPM gene,
and this shows a schematic of the structure of the ASPM
protein between humans and ferrets and mice.
And as I told you, the mice has just a shorter encoded protein,
and the majority of this protein is
encoded by these repeated domains, which are called
IQ domains or IQ repeats.
And they're just called IQ because every one of them
starts with an isoleucine, which is abbreviated with an I--
that's an amino acid--
and glutamine, which is a different amino acid
abbreviated Q.
But when we cloned this gene, Jeff Woods
made the joke that this is how we
know that God has a sense of humor,
because could it actually be possible that the number of IQ
domains specifies your IQ?
Could it actually be that easy that evolution had it that way?
But anyway, clearly, not only are the stem cells different
in mice and humans, but also, the structure of this gene.
And if you actually look at the relative conservation
of the ASPM gene, here it shows between humans and chimps
and gorillas, you can see that, in fact, rodents
are a real outlier with a very low level of similarity,
suggesting the gene is most different between rodents
compared to other mammalian species.
And so what we wanted to do is, again,
to try to get at the cellular biological mechanism
of this gene.
We wanted to create an animal model that
was more close to the human.
And as I mentioned, the drosophila has only 21
of these repeats.
And so actually, most of the loss of these repeats
in the mouse is due to a deletion of just one
little spot of the genome--
in some ways, a single evolutionary event
that actually removes many of these IQ repeats.
So originally, we thought, in fact,
that the mouse version was like the ancestral
version to the human that the human gene grew.
It turns out, actually, that the human
has the conventional version for mammals
and that mice actually seem to have gone out of their way
to shorten the protein to make a smaller encoded protein,
that that is actually the evolutionary event that's
actually relatively unique to rodents.
And so for this reason, then, we created an animal model
in these ferrets, because these days,
you can knock out a gene in anything.
You've heard about CRISP-R and all this stuff.
And so we thought, well, we'll just make a large animal model.
It happens that ferrets are convenient to work with.
They breed very easily in captivity.
They have huge litters.
They're very easy to study developmentally,
and so we targeted it in ferrets.
And here, you can see that they make a much better model.
Compared to humans, the brain is reduced by about 40%
of its size.
In fact, it has a defect much like the humans.
What actually happens in the human brain when you lose ASPM
is that the brain doesn't--
the cortex doesn't become thinner.
It becomes smaller in surface area.
So the thickness is normal--
about 100%-- but the area is smaller
so that the cortex is reduced in size.
And the same exact thing happens in the ferret,
where in fact, the surface area is greatly reduced in size,
but the thickness is barely reduced in size at all.
And in fact, it's an amazing thing about this ferret
that the very parts of the brain that
are thought to be most recently evolved,
like the frontal cortex, and the corpus callosum,
and the lateral cortex, are those
that are most severely affected by the mutation.
And then, parts of the brain that we
think of as very ancestral that are shared by reptiles
and so forth are barely affected by this mutation.
And so the mutation actually causes a fairly simple change
in the cortex that I'll just show you about.
This is a section of a newborn ferret,
which is in the middle of the process of making its neurons.
So their stem cells are down here.
The neurons are collecting up here.
And so the stem cells are illustrated here in color.
The neurons are collecting up here, but you can't see them.
And what happens when you lack ASPM
is that you have a shift of these stem cells to these guys.
You see that they all collect in this big clump, which
you don't see in the normal.
And these Outer Radial Glial cells, or ORGs,
are formed precociously.
And so it appears that then ASPM is also
a slightly different kind of switch that
controls this change from this kind of stem cell
to a slightly more differentiated or mature stem
cell that has less in the way of proliferative properties.
And so the loss in both species--
it reduces the cortical surface area
without really changing the cortical thickness.
We think that basically, these guys, the red ones,
control the surface area.
They divide and they end up next to each other and next
to each other, and they expand the surface area of the cortex.
And then, these guys, once they leave the ventricle, in fact,
they generate the different layers of the cortex above.
And so there's a sequence of development,
and ASPM affects this step and that step mainly.
And so this is still a hypothesis
that tweaking ASPM, changing it in subtle ways
might regulate the size of the brain,
but it's certainly-- all of the biology
points in that direction.
But then also, it suggests this thing about mice.
Again, we think of mice as the prototypical lab model
for humans and that they're sort of a proto-mammal.
But in fact, now I think of mice as exquisitely selected
by evolution, that in fact, the size of the brain for a mouse
works perfectly for a mouse because mice are ruthlessly
evolved to be able to crawl into tiny holes
that a ferret can't fit into.
And so they want to have a small head because it's
the size of the head that actually determines
the size of the hole you can crawl into.
And in fact, if their heads were any bigger,
then they would be eaten.
Or it's something like that.
Perhaps it's a bit simplistic.
But in fact, it seems that mice don't
want to have a smaller brain because again,
the entire rodent order has disrupted ASPM
to a certain extent.
So now, the last kind of genomic change that underlies evolution
is probably the most common but is the hardest to study,
and this is changes to the dark matter
of the genome, the non-coding parts of the genome.
And so this is a part of the genome
where there's no genes there.
It just seems like it's a spot in the middle of nowhere.
And what is in the dark matter of the genome?
Well, some of it is true junk, but a lot of it
are on/off switches, are regulators
that tell the genes near them, in fact,
where and when to be expressed.
And so they control the patterns or the levels
of expression of nearby genes.
And so I'll just tell you a little bit
about how we're trying to get at the function of some
of these non-coding parts of the genome.
And we've been focusing on a particular subset
of these non-coding parts of the genome that have been already
identified by a variety of different laboratories,
and they're referred to as human accelerated regions.
And accelerated just means they show
accelerated change in the branch of evolution
that leads to humans.
And so this is an example of the genome
of a mouse, a ferret, a rhesus, a gorilla
lined up and compared.
And a part of the genome that is neutral, your average genome,
will have a certain number of substitutions
if you compare one species to another,
and the human will have about the same number
of substitutions relative to any other given species
as anyone else, to one another.
Now, there are other parts of the genome that
are intensely conserved, that are identical or almost always
identical or almost completely identical
in a large number of different mammals.
And when you compare genomes and you see this conservation,
this is nature's way of telling you
that a sequence is really, really important,
and that it does not tolerate any sort of change,
and that any change is very likely to be damaging.
And so you can identify these sequences of the genome
as having essential functions just by virtue
of the fact that they are so highly
conserved between species.
And then there are the human accelerated regions.
Human accelerated regions are defined
as parts of the genome that are highly
conserved in many, many different species,
but then are a little different in humans
compared to other species.
And so this conservation between many species
suggests that they have essential functions.
And then the changes between humans and other species
can have a variety of explanations,
but one of those explanations might
be that that essential function has
changed just a little bit between humans
and other species.
It can also mean, of course, that, in fact,
an essential function has lost its essential function,
has become garbage.
And so that's why all of these things have been introduced.
So we need additional information
to prove which of the human accelerated regions
are really important, by trying to explore
what the actual essential functions of these HARs are.
Now, we know that for many of these HARs,
they've been identified by a variety of experiments
as actually being sequences that direct patterns of gene
expression.
And in some cases, you can even identify HARs
where the human version of the HAR
directs a different pattern of gene expression
than the chimp version of the HAR, and here's an example.
This is HAR number five, and the chimp version--
which PT stands for chimp, Pan troglodytes--
that drives expression, but at a relatively low level
in this little blue dot in this embryonic mouse.
And this happens to correspond to
the embryonic cerebral cortex in that developing mouse.
And then, if you take the human version,
you can see that the expression is really, really juiced up
in the cerebral cortex and spreads
to other parts of the back part of the brain
and the spinal cord.
And so in fact, the evolutionary changes
in the sequence of this particular HAR
seem to confer a change in function.
The problem is there are more than 3,000 spots
in the genome that have been identified as HARs.
Each one of these HARs is about 100 to 300 base pairs long.
Sometimes they're in genes.
Most of the time they're in the middle of nowhere,
and we don't know that much about what
they're connected to.
And so the challenge is to figure out whether all 3,000
of these are important to making us human
or whether some of them are more important than others.
And so we wanted to ask the question
whether we can identify HARs that have essential functions.
We thought, well, OK, humans have social structures that
look a little different than chimpanzees,
and so there must be sequences in the genome that
regulate those changes in social structure.
And so if HARs are important in defining us as human,
then some of them should have essential neurological
functions.
So if we look at patients that have a very severe disruption
of a HAR, maybe they have a loss of function
that might tell us about what that particular HAR is doing.
And by identifying those HARs that
have essential genetic functions in humans,
we can then winnow down this list of 3,000 HARs
to try to identify those that might be most important.
And so a couple of years ago, we showed
that indeed, some of the HARs can
be disrupted in kids that have autism
or intellectual disability.
This is a particular HAR, number 426,
and we found two different families
that had autism and intellectual disability where
they, in fact, had disruptions of this HAR 426.
And HAR 426 we found out connects
to a gene which is called CUX1.
CUX is similar to another fly gene called Cut.
And CUX and its related gene, Cut--
they also are sort of switches, but they
don't control stem cells.
They control neurons so that in flies, if you lose Cut,
you take a complicated-looking neuron and it gets simpler,
or if you get too much Cut, it takes a simple-looking neuron
and makes it more complicated.
So in fact, Cut controls the elaboration
of the patterns of connectivity of neurons,
and the same thing happens to be true--
oops.
The same thing is true of the mouse version,
that if you lose it, you take a complicated neuron
and you make them simpler.
If you overexpress it, you take a simpler neuron.
You make it more complicated.
So again, nature seems to have very carefully titrated
the exact right amount of Cut for the shape and size
of the neurons that it wants.
And in fact, interestingly, Cut is expressed only
in the upper layers of the cortex,
layers two, three, and four.
These happen to be the layers that
are most highly elaborated in humans
and which are very simple in rodents, and even missing
in some of the smallest mammals like hedgehogs.
So human brain evolution, then, is likely
a tinkering at many different sites in the genome.
This is an idea first developed by Francois Jakob, now 40
years ago.
There are probably many sites in the genome.
There are probably many mechanisms, but a finite number
of mechanisms.
It's a big problem, but it does seem
to be a finite problem because the genome is,
after all, only 6 billion base pairs.
And so non-coding as well as coding gene evolution
is involved.
As I say, at this point, we have too many candidates for sites
that might be involved.
The key is to narrow them down to which
ones are most important.
Now, what proportion of these are central to the brain,
and which proportion have evolutionary roles?
So I'm running out of time, but I still
have a couple of minutes to just briefly outline what we're
trying to do with the Center.
One thing we're trying to do is create the world's largest
resource of DNA sequence of ancient and archaic humans.
This is the work of David Reich, and he'll
be coming to speak to you soon.
And this sequence allows us to do
more kinds of analyses to identify
these human-specific changes, or actually
to find some of the sequences changes
that people have claimed are human-specific
and find that, actually, maybe they're not so important.
And then, we also want to study these aspects of learning
and memory that involve changes in gene transcription
to try to understand some of these that might
be evolutionarily dynamic.
And we feel that we can't help but learn about genetic brain
diseases of children as a part of doing this work because not
only is it important to identify the causes of childhood brain
disease, but this is, in fact, how
we identify the sequences in the genome that are essential.
Just as a biologist, if you want to understand what's
essential in the human genome, you
can only do that, in the case of humans, by studying disease,
because that really tells you what matters in the genome.
So like I said, David is going to be talking about large-scale
sequencing of these old bones that get dug up that are 5,000,
10,000, 50,000 years old, and he's developed ways
of purifying the DNA--
the very small amounts of DNA-- and sequencing
it to create the largest resource of ancient DNA.
And in fact, David's lab has generated more than half
of the world's collection of these ancient genomes.
And so having this larger DNA sequence resource
capturing more of the diversity of humans--
we can capture not only the diversity of living humans,
but we can capture the diversity of humans
which are no longer living.
We can get the broadest sense of what
sort of range of the genome is consistent with being
a normal human.
We can then identify the various changes
that occur at each stage of the evolutionary tree.
And then, as I said, we also want
to identify physiologically in the genome, what
are the parts of the genome that regulate aspects of learning
and memory and this control of gene transcription
that I told you about that's so important-- the control,
for example, of the levels of that Cut gene?
And that's where Mike Greenberg is taking the lead,
because this has been the mission of his lab
for many years.
And here's an example.
He found a gene called Osteocrin, which is expressed
in the bone in the mouse.
That's why it's called Osteocrin.
But in fact, in the humans, it's actually
expressed very highly in neurons in relationship
to their activity.
So when neurons are active or depolarized,
their levels of expression of the Osteocrin
go way up in humans.
And in fact, if you take a monkey and you close one eye,
the cells that are responding to the open eye
express high levels of Osteocrin,
and the cells that are not active
because they're exposed to the closed eye in fact
show low levels.
But this is a gene that has totally
changed its function between mice and humans.
It's not a bone gene anymore in humans.
It's a brain gene.
And so this is another way of identifying
genes that might have dynamic evolutionary effects.
And then, like I say, we'll use the genetics of pediatric brain
disease to parse out which of these evolutionarily dynamic
sequences actually have essential functions.
And we have to still do animal models,
but we'll need some new animal models, things
like not just mice, but ferrets or even potentially other
animal species as well.
So thank you very much for your attention.
I'm just going to acknowledge some of the people.
The ASPM work in my lab was done by two postdocs--
actually, now both former postdocs.
The HAR mutation work was done by Ryan Dunn,
a postdoctoral fellow in the lab.
There are many other people that contributed that--
I don't have time to acknowledge all of them,
since this is intended as sort of a general overview talk.
But again, thanks very much for your attention.
I'll be happy to answer questions.
[APPLAUSE]
-------------------------------------------
Classical Architecture, Warm and Lightly Joshua Tree Cabins - Duration: 3:55.
Classical Architecture, Warm and Lightly Joshua Tree Cabins
-------------------------------------------
Nesha - Hindi Version | Arman Alif | Yaar Mere Mujhko De R Joy |Latest Sad Song 2018 NS Music - Duration: 3:52.
Nesha - Hindi Version | Arman Alif | Yaar Mere Mujhko De Itna Tu Bata | R Joy |Latest Sad Song 2018
-------------------------------------------
Unused Railroad Exploration - Duration: 4:43.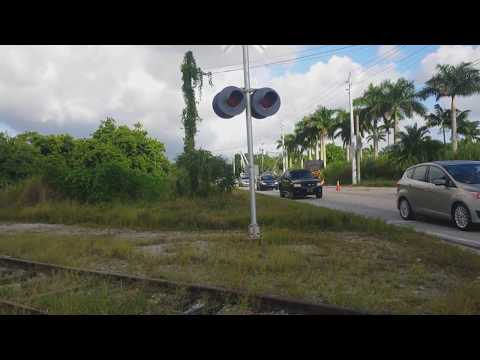
hello ladies and gentlemen, RailROL82 here. Your railroad archaeologist so
I'm over here at the CSX Homestead subdivision on you I tell you Rico drive
processing I did a tour of this crossing last year and today I'm gonna show you
what this time looks like now that it's officially out of service here we can
see some girls on it so Moodle right there
this thing was built this is facing south west facing north east the sign
was built in 1927 looking for a real with the date on it 75 pound rail built
by a Marilyn Marilyn steel I do not see a date though but yeah I know for a fact
that was built in 1927 and you can see the old wooden ties and if I stand on
this line okay it's mostly gross just see what it feels like to have been on
this line this is what it was what it would have felt like see a nice
residential area next to here and agriculture it's on my right I'm gonna
include a little map into that crossing so you guys can know where I'm at
and this is facing north east so you see over here we can find a date on the
rails yep 1926 right there 1926 built by the
seaboard airline railroad design came from my Annie from 5-9 the International
Airport and do you ignore that it came to haul away from from Central Florida
to homestead homestead is a city that is about a few miles south of here just to
put it into perspective on this Yurika drive here this crossing is 1
84th Street and this line ends at around 320 something 330 something Street so
this is our about 20 miles away or so from the end of the line
you can see these rails
pure rust
that's a shame because if these reels could talk I'm sure they'd have some
very interesting stories to tell
and yeah there we see the gateless crossing it's really case on the other
side so yeah guys that's this crossing and I'm gonna continue further south to
Seoul I thank you for coming along with me please subscribe for like dinking
please comment over and out
-------------------------------------------
Иностранцы слушают баттл-реперов Slovo Spb - Duration: 7:23.
-------------------------------------------
Bài Lỗi Ô Vuông, Bài Thửa, Bài Dấu, Bài Điểm, Bài Công Lỗi Công Ty Các Loại - Duration: 6:16.
-------------------------------------------
[PREVIEW] "COMBAT" : BTS IMAGINE - Duration: 1:03.
The doctor
He told us she was dead
That she didn't suffer
She was smiling
A lot
Our angel
She was adorable
I can't believe that she isn't here anymore
"COMBAT" // eng : fight
coming soon
-------------------------------------------
A l'occasion de la fête nationale grecque - Duration: 5:53.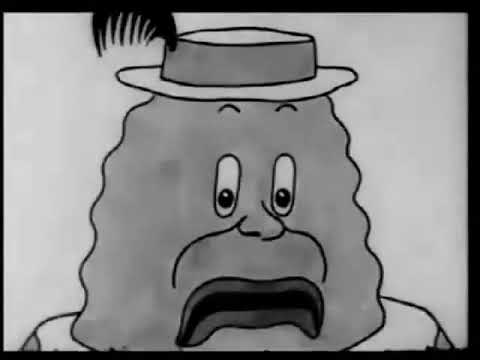
-------------------------------------------
Seat Leon 1.6-16V Last Edition AIRCO - Duration: 1:07.
-------------------------------------------
Tutu for Girls, parties and costumes - Duration: 11:34.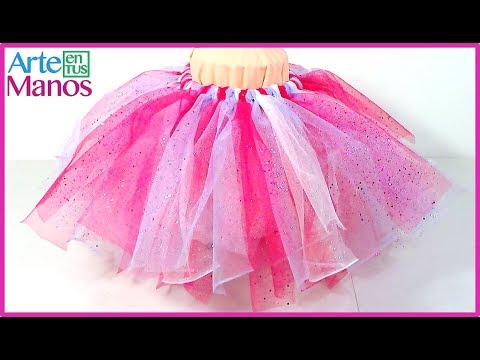
Hello friends, welcome to your channel Arte In Your Hands for today we are going to share
a simple project, this month, is the month of children or the month of the witches
as we say here in Colombia or as they call it in other countries halloween
We were looking for a tutu because We wanted to disguise a girl
unicorn that is very fashionable then we went to look for a tutu and there are some
very simple and very economical but also when we were looking for a little more
elaborated were too expensive so we decided to do it ourselves
I invite you to join me perform this tutu I hope you like it
put it into practice and give us your "Like" share it and subscribe
also if they have not done it there yet the little red button also remembers
activate the bell to get you all our notifications and if
you want to accompany this tutu with the unicorn diadem we make you
I also invite you to visit the video here in the description I'm going to
leave the link so you can do it, not being more then welcome!
and for this simple project we need then spring or elastic
approximately 2 centimeters, needle, scissors and the tull that is the
protagonist to make this tutu, this tull let's choose three tones that are
white, fuchsia and lilac then here is I've already cut them all have a thickness
of 10 centimeters and the length in this tutu is 75 centimeters if the girl is
bigger or smaller that measurement varies to that of the 75
centimeters vary this is for a girl approximately 10 years or so
that if it's smaller then it's less long there are about 65 or 60
centimeters or if it's bigger then it's no longer 75 but it can be
about 90 cents if you have in tell the age and size of the girl the
elastic is the same you have to take the measure to the contour of the waist and
considering that then also is the amount of elastic
the minimum they buy a meter take the measure and the remainder they keep it
for another project, here the first thing that let's do is start joining
the elastic will adjust so that we stay strong
well here then it is already perfectly so stay strong and now we're going
to start taking the pieces each It measures 75 centimeters from here here 75
10 centimeters wide what we are going to to do is to fold it in the center that we
be even and we will put it over
of the spring and let's do as a kind of ring and this ring we're going
to start going through there the same tull what let's braid it can
we are adjusting it
that it fits well darned well in the top that's what makes the
tutu when you do not have it totally already ready to be well filled well pompous
then this is a tull illusion is smooth it's very good to work it these are
very cute they are frosty but they are thicker heats your hands a lot
then be careful with going to get wet then to work on this project then equal
75 centimeters long and is wide he's going to put it on the elastic doing
the cavity and then can enter
as I tell you how thick it is then it's a little harder to
work but the result is worth it then we are organizing
as we are working on him is giving way
and one white in purple or purple and now let's introduce one pink
strong or fuchsia or whatever the color that deciding here I'm just
giving an idea
I always try that the spring does not lose its form the idea is that always
is right and it's up to us to make an effort in working the tull this is thick
because the one that is thinner with this It is easy to work
and we repeat then another one of color white then again in lilac and then
the fuchsia
so until you finish the whole short you have to white is much easier
because I found it thinner
but this being so frosty are very cute
well here then I already have advance how are we going with the project, look what's going
the same sequence
I keep doing the same for work repetitive
what are we going putting the tulle
and I really do not know how they say in other countries in Colombia it is called tulle
illusion tulle, frosty blue with what they also make many mosquito nets (mosquito nets) or ornaments
for babies' rooms but now we already find it that many colors
decorations that makes it very cute
and now with the fashion of the tutus then it's coming
prints too
and so we do we keep working until finish
look here is the union when we made all this tissue in
he is not going to notice he is not going to see forever
that is very tight, very pegadito one piece of the other so that it is more
pompous to make it more full and the skirt will look much more and much more
big much more
remember to always make the ring and for here I introduce the fingers and sack
and this same process we do already finish
well here I am placing then already the last piece
if suddenly in a moment they get to see what
they lack that they have space because of soon it is not as full as they want
very carefully begin to go running the pieces
and they start doing all the fabric with a correct tint
the idea is not to put another tone run we fit well assorted
the spaces and then there yes no more than ever in the same sequence
at least in this one we did fuchsia white and lilac fuchsia white for
that he always plays the game and that pretty as it looks
so then it is now you can take them some photos to see how it is already
the approach well as it looks we already put it as a skirt or as a tutu
I hope the project likes it put into practice that is helpful and
see you in a next video here in your channel Art in Your Hands
No comments:
Post a Comment